A major bottleneck limiting the advancement of energy storage and conversion technologies is the development of multifunctional, selective, and highly conductive membranes and solid electrolytes.
Polymer-based electrolytes are amongst the most promising materials for next-generation applications due to their high electrochemical stability, mechanical flexibility, processibility, and their ability to maintain electrical contact over large temperature variations (good adhesion properties) better than their more brittle ceramic counterparts. Furthermore, they provide an ideal platform to support next generation energy storage architectures, such as those relying on the interdigitation of an electrolyte with the electrode. A grand challenge lies in the fact that polymer membranes, however, have yet to achieve the necessary conductivity and selectivity for fast ion transport and suffer from water management issues that restrict operating temperatures due to the need for water (i.e., hydrogen bonding networks) to transport protons. Efforts to improve conductivity, mechanical modulus, flexibility, and safety, by combining polymers and superionic ceramics, have thus far failed to provide the necessary conductivity.
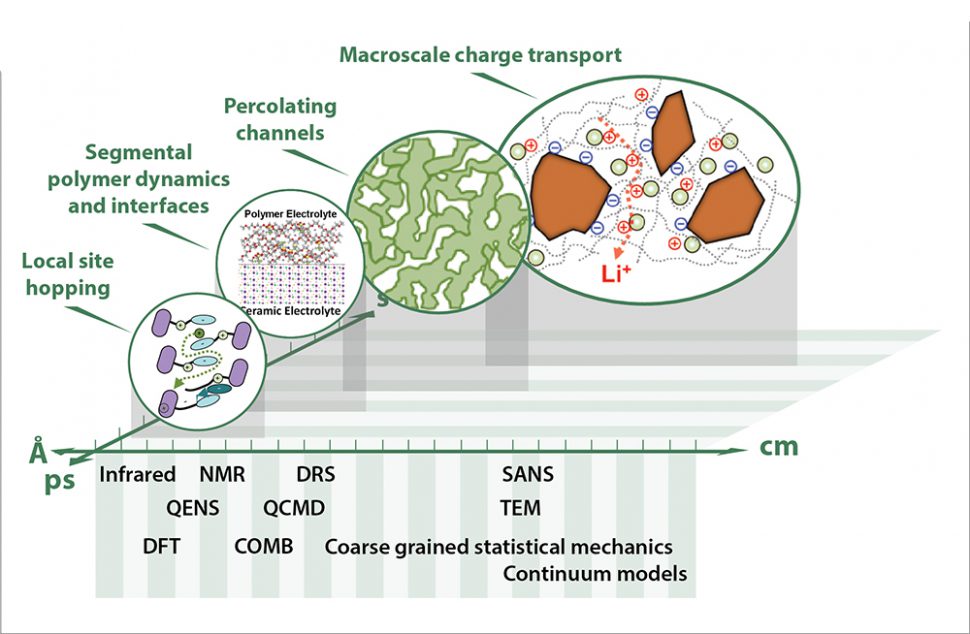
This center seeks to build a predictive, data-driven, physics-based mechanistic model of ion and proton transport in polymers and polymer-ceramic composites to enable the targeted design of next-generation energy storage and conversion materials. Materials-specific experimental and computational data covering a broad range of length and time scales will inform macroscale descriptions of charge transport to accelerate the design of polymer electrolytes with fast, correlated ion transport. A foundational goal is to understand the nanoscale origins of ion and proton conductivity by identifying the mechanisms that determine how ions and protons move in polymers and composites on a microscopic (0.1–10 nm) scale and to correlate these movements to macroscale transport properties. In essence, we seek to discover new systems with superior charge transport to what is available today. Emphasis will be placed on determining (i) how ion diffusion/hopping can be decoupled from polymer segmental dynamics and how to significantly reduce the energy barriers for charge (alkali ions and protons) hopping in polymers; (ii) how conductivity is enhanced by specific ion channel morphologies and polymer solvation microenvironments; (iii) how ions move along or across dissimilar interfaces; and (iv) how specific chemical additives can accelerate charge transport and how they can be introduced in microstructured channels and at interfaces to improve conductivity. This will enable us to (i) increase ion mobility, (ii) improve ion solvation and dissociation, (iii) devise new chemical systems for fast hopping transport, and (iv) promote fast, correlated charge transport in a material across nanometer to millimeter length scales. This research will concentrate on (i) small classical cations (Li+ and Na+) diffusing through a matrix, and (ii) protons where hopping transport and dynamic hydrogen bonding can be leveraged. As model polymer systems, we will initially focus on single ion conducting polymers and polymer-composites relevant to future clean-energy technologies. Single ion conductors provide selective ion transport and have significant advantage for many applications, in comparison with dual ion conductors. These systems are chosen to allow for one-to-one, seamless, comparisons, across multiple length and time scales, between theory and experimental synthesis and characterization efforts. To achieve our goal, FaCT is built upon two thrusts and a crosscut: